- 28 Oct 2024
- 7 Minutes to read
Combustion characteristics
- Updated on 28 Oct 2024
- 7 Minutes to read
Flammability properties
In the previous chapter, some fundamental principles of fire development are introduced. To understand the nature of fire development and spread, this chapter will explore the concepts of flammability and flame characteristics. Different fuels are characterised by differentiating flammability properties. Flammability depends on several factors:
Flashpoint: This is the lowest temperature at which a liquid fuel generates enough vapour to form an ignitable mixture with air.
Energy content: This factor helps us understand the potential amount of heat released during combustion.
Flammability limits: These limits determine the minimum and maximum concentration of flammable material at which combustion will occur.
The flash point and flammability limits are defined through specific experimental approaches. For flaming combustion to begin and continue, the concentration of fuel vapour in the burning mixture (oxidant and fuel) must fall between specific ranges called Lower Flammability Limit and Upper Flammability Limit for that fuel. Additionally, the ignition source must be sufficiently robust to initiate combustion reaching Minimum Ignition Energy.
Lower Flammability Limit (LFL) | The concentration below which too little flammable gas is present in the air to sustain combustion. |
Upper Flammability Limit (UFL) | The concentration above which too little oxygen is available to sustain combustion. |
Minimum Ignition Energy (MIE) | The lowest energy required to ignite a flammable material in air, oxygen or other oxidant. |
These definitions apply universally to the gaseous phase. However, for dust originating from solid materials, parameters such as particle size, distribution, and dispersion in the gas play crucial roles in combustion. These factors are sometimes incorporated into the Fire Triangle.
The plot of the concentration of flammable vapours versus temperature illustrates the interrelation of the definitions above. Some key observations include:
Typically, with increasing temperature, the UFL increases, and the LFL decreases.
The LFL intersects the saturation vapour pressure curve at the flash point. At this lower limit temperature, the saturated vapour is just rich enough to ignite.
The autoignition temperature signifies the lowest temperature within an autoignition region.
A plot of the concentration of flammable vapours versus temperature
Flammability properties are typically known for pure chemicals, but many fuels, such as hydrocarbons, are usually mixtures of multiple pure chemicals. Precision in determining the LFL and UFL is achieved through experimental measurement conducted in laboratory conditions, ideally mirroring process conditions. However, several available methods provide approximate estimations for the flammability limits of vapour mixtures. For example, LeChatelier's rule can be employed to estimate the LFL and UFL of vapour mixtures (Perry, 1997).
For further understanding of flammability limits, the flammability diagram may be incorporated. The flammability diagram emerges as a pivotal tool, providing a precise graphical representation that delves into the intricate relationship between the concentration of flammable mixtures and flammability. The flammability diagram primarily focuses on the composition of flammable substances within mixtures, commonly involving air, oxygen, and inert nitrogen and illustrates the concentration in volume % of a flammable substance within a mixture, typically in the presence of air. The fundamental components of a flammability diagram include the LFL, UFL, and the flammability zone.
Apart from flammability limits, other material-specific properties like "heat of combustion ΔH c", "laminar burning velocity" (gases) and "burning mass flux" (liquids) play an important role in the combustion behaviour. The "heat of combustion" describes the amount of energy being released upon combustion. The laminar burning velocity, dependent on the concentration of the vapour, describes how fast a flame can travel through a flammable cloud, and is significant for the potential explosive strength of a confined vapour cloud explosion. The burning mass flux expresses how rapidly a liquid pool may burn.
Fire development and spread
Fire development stages
There are four fundamental stages in fire development:
Incipient ignition phase: This initial stage is characterised by the heating of potential fuel sources.
Growth stage: Following this incipient phase, fire development enters the growth stage, marked by visible flaming combustion.
Full development and flashover: As the fire intensifies and reaches temperatures of around 600 °C, it attains full development within the compartment, triggering the "flashover" condition and initiating the burning period. This severe stage witnesses elevated temperatures and heat flux, causing all exposed surfaces to burn, with ventilation governing the heat release rate and significant effects on building elements.
Decay period: Subsequently, the fire enters the decay period as the fuel becomes exhausted, marking the conclusion of the natural fire cycle (Uche, O and Muhammad, I., 2014).
Additional insight from the figure below reveals key phases such as initiation, characterised by an exothermic reaction with elevated temperatures, fire development influenced by fuel and outdoor conditions, flashover as the transition to a fully developed fire, and burning out as the final phase with diminishing temperatures and exhausted flammable substances. These stages provide a framework for understanding the progression of a fire and are crucial for implementing effective fire prevention and suppression strategies (Erdélyiová, R., Figuli, L. and Ivančo, M., 2020).
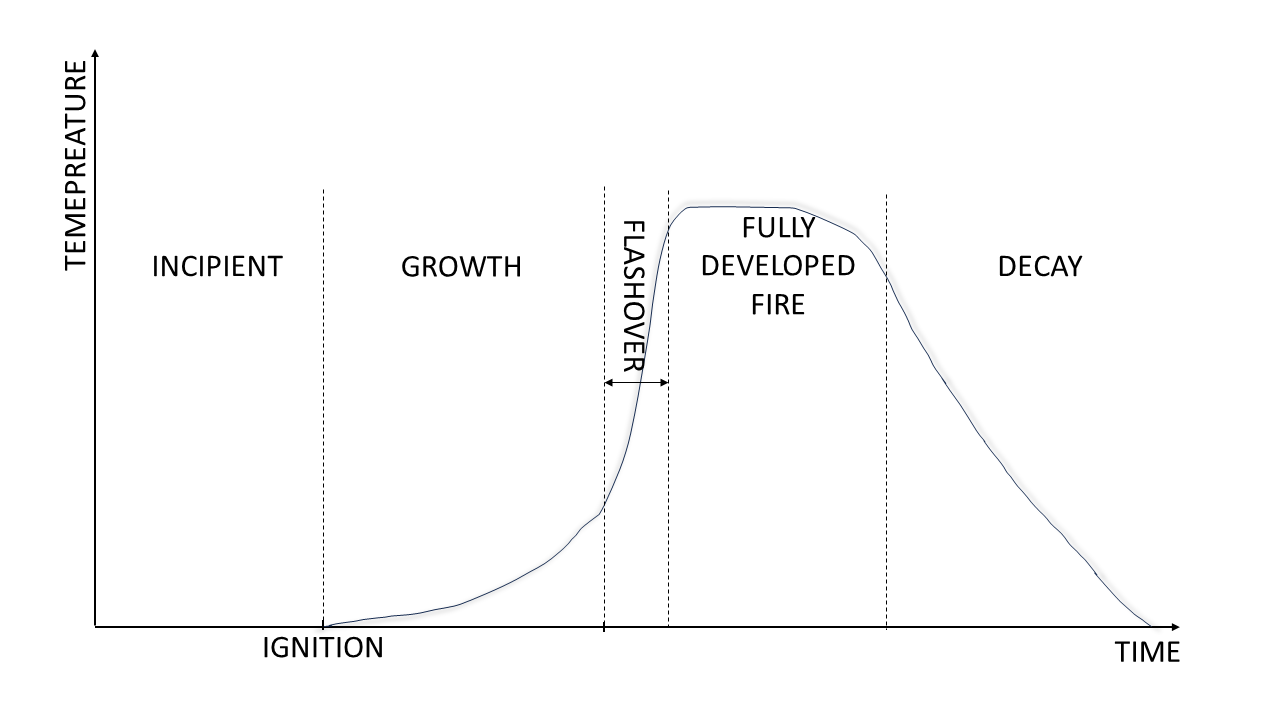
Fire development stages
Differentiating features of flame zones and their implications
Flames are commonly divided into three zones:
Ignition zone | Combustion or reaction zone | Post-combustion or exhaust zone |
---|---|---|
This is where the combustion process begins. Fuel and oxidiser mix and reach a temperature at which ignition occurs. Typically, this zone has a lower temperature compared to the other zones. | This is where the actual combustion reactions take place. High temperatures are maintained, and both fuel and oxidiser are actively reacting to produce heat, light, and other combustion byproducts. | This is the region where the combustion byproducts are cooled down and expelled. |
Temperatures decrease as the products move away from the high-temperature reaction zone while soot formation may reduce the radiative energy from the flame in this zone.
Flame Propagation
Flame propagation refers to the spread of fire through a fuel-air mixture, influenced by factors such as fuel type, concentration, and environmental conditions. Depending on the speed of flame propagation we can distinguish between:
Laminar flame propagation: Characterised by a thin flame front with a smooth surface that can be curved. Laminar flame propagation is slow, with hot combustion products expanding ahead of the flame.
Turbulent flame propagation: Characterised by a rough combustion zone.
There are 2 basic flame propagation mechanisms in which laminar and turbulent flame propagation can take place and are closely interconnected to the explosion mechanism. Therefore, these aspects will be discussed in detail in the explosion chapter Detonation, Deflagration and DDT. (Bosch, 2005)
Heat transfer from flames
Heat transfer plays an important role in fire growth and spread, occurring through three processes: conduction, convection, and radiation.
Conduction | Convection | Radiation |
---|---|---|
Heat transfer through collisions between neighbouring atoms or molecules | Heat transfer by movement of a heated fluid such as a gas or a liquid. | Heat transfer from the movement of charges in the material that is converted to electromagnetic radiation. |
Heat flux
In further distances, from fire, the heat radiation is predominating in the heat transfer. Heat flux is determined by factors like the Surface Emissive Power (SEP), view factor, and atmospheric transmissivity.
SEP: Represents heat flux due to radiation at the flame’s surface area in W/m 2. To determine SEP, the flame temperature needs to be known, which is difficult to determine, therefore a so-called ‘‘solid flame’’ approach is frequently used, which assumes that the heat is radiated through the visible flame surface area although the flames do not emit radiation from their surface area only.
View factor: Describes the overall viewing angle of the radiating surface towards the receiving plane. This factor incorporated the flame dimension and shape and relative position and orientation of the receiving object.
Atmospheric transmissivity: Accounts for the radiation absorbed by air between the flame and the receiver (Bosch, 2005).
Conclusion
An in-depth understanding of flammability properties is paramount for comprehending the dynamics of fire development and spread. Through exploring principles such as flash point, flammability limits, and minimum ignition energy, one can discern the critical factors influencing the initiation and propagation of combustion. The interplay of parameters like lower and upper flammability limits elucidates the delicate balance required for sustained flaming combustion. Moreover, the delineation of flame zones sheds light on the intricacies of the combustion process, from ignition to post-combustion phases. Flame propagation mechanisms further underscore the importance of fuel type and environmental conditions in dictating the speed and nature of fire spread. Furthermore, heat transfer mechanisms elucidate the diverse pathways through which fire exerts its influence, from conduction and convection to radiation. Ultimately, a nuanced comprehension of flammability properties not only deepens our insight into fire behaviour.
Reference
Bosch, C. v. (2005). Methods for the calculation of physical effects 'Yellow book' CPR 14E. The Hague: Ministerie van Verkeer en Waterstaat.
Perry, R. H. (1997). Perry’s Chemical Engineers’ Handbook. McGraw-Hill: New York.
Uche, O and Muhammad, I. (2014). Probabilistic Sensitivity Assessment of Composite Steel Beam Exposed to Fire. American Journal of Civil and Structural Engineering. 1. 55-61. 10.12966/ajcse.07.03.2014.
Erdélyiová, R., Figuli, L. and Ivančo, M. (2020). Prediction of fire loading on the structures using computational fluid dynamics. MATEC Web of Conferences. 313. 00033. 10.1051/matecconf/202031300033.